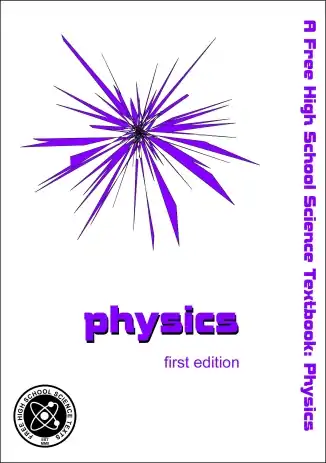
About FHSST
Free High School Science Texts (FHSST) is an initiative to develop and distribute free science textbooks to grade 11 - 12 learners in South Africa.
The primary objectives are:
- To provide a *free* resource, that can be used alone or in conjunction with other education initiatives in South Africa, to all learners and teachers
- To provide a quality, accurate and interesting text that adheres to the South African school curriculum and the outcomes-based education system
- To make all developed content available internationally to support Education on the largest possible scale
- To provide a text that is easy to read and understand even for second-language English speakers
- To make a difference in South Africa through helping to educate young South Africans
FHSST Website - FHSST Physics on Wikibooks
Other FHSST books on Wikibooks:
- FHSST Biology
- FHSST Computer Literacy
- FHSST Chemistry
Introduction
<< Main Page -- First Chapter (Units) >>
Introduction
Physics is the study of the laws which govern space, structure and time. In a sense we are more qualified to do physics than any other science. From the day we are born we study the things around us in an effort to understand how they work and relate to each other. For example, learning how to catch or throw a ball is a physics undertaking.
In the field of study we refer to as physics we just try to make the things everyone has been studying more clear. We attempt to describe them through simple rules and mathematics. Mathematics is merely the language we use. The best approach to physics is to relate everything you learn to things you have already noticed in your everyday life. Sometimes when you look at things closely, you discover things you had initially overlooked.
It is the continued scrutiny of everything we know about the world around us that leads people to the lifelong study of physics. You can start with asking a simple question like "Why is the sky blue?", which could lead you to electromagnetic waves, which in turn could lead you to wave particle duality and energy levels of atoms. Before long you are studying quantum mechanics or the structure of the universe.
In the sections that follow notice that we will try to describe how we will communicate the things we are dealing with. This is our language. Once this is done we can begin the adventure of looking more closely at the world we live in.
Units
Introduction
Science focuses on studying how things happen in the real world—things you can see, touch, hear, smell, feel, or imagine such as time.
Fractions and decimals
Early society had primitive ideas such as one, two or many, then more sophisticated means of counting emerged, mainly relating to trade. Units of weight, volume and money were at first integer whole units, often recorded by notches in a tally stick or marks in clay tablets. About 6000 years ago, with the advent of writing came units such as the length of the king's arm, together with the idea of multiples such as dozens and scores, together with vulgar fractions based on halves, quarters and so on., In order to describe these things, it is necessary to carefully measure what is observed.
In 1791, following the French Revolution, the decimal system was published based on tens and multiples or fractions of ten. The idea of ten months per year and ten days in a week were quickly dropped, but for most purposes it was revolutionary, with integrated standard weights and measures, such as fixing the second to the length of a pendulum of one metre, and the weight of a kilo of water the same as a litre volume. Since the 1960s the International System of Units ("Système International d'Unités" in French, hence "SI") has been almost universal outside the United States of America, which still prefers a version of the Imperial Roman measuring system which emerged more than 2000 years go and which is complicated and illogical.
Measurements must always be reported with appropriate units, which specify what type of quantity is being discussed - weight, length or whatever. For science and engineering, the SI system is universal and is not 'owned' by anyone, so it remains constant and free of political manipulation. As recently as 1897 the Indiana State Legislature,attempted (unsuccessfully) to set the value of Pi to 3.2, and during 1940, in Britain, the weight of a pound (lb) loaf of bread was legislated at a lower weight to concerve supplies during food rationing, giving rise to the expression 'baker's dozen' because you needed thirteen new loaves or buns for the same quantity of bread as 12 of the correct weight!
The Measurement and the Decimal Metric System
As a simple example of the importance of units, imagine you had to make curtains and needed to buy material. The shop assistant would need to know how much material was required. Telling her you need material 2 wide and 6 long would be insufficient—you have to specify the unit (i.e. 2 metres wide and 6 metres long). Without the unit, the information is incomplete and the shop assistant would have to guess. If you were making curtains for a doll's house the dimensions might be 2 centimetres wide and 6 centimetres long!
It is not just lengths that have units. Any measurement of any physical phenomenon—time, temperature, force, or voltage, just to name a few—has units.
Tip: Many physics problems ask you to determine a specific numeric quantity. When you solve the problem, do not forget to specify the units of your answer: even if you have the right number, your answer is not correct unless you include the correct units.
SI Units
In the remainder of this class we will be using SI units, which are defined in the table below. These seven units are used to measure fundamental quantities, and are the basis of everything we will do, as will be discussed in more detail in the next section.
Base quantity | Name | Symbol |
---|---|---|
length | metre | m |
mass | kilogram | kg |
time | second | s |
electric current | ampere | A |
thermodynamic temperature | kelvin | K |
countable amount of substance | mole | mol |
luminous intensity | candela | cd |
SI Units (Système International d'Unités)
These units are internationally agreed upon and form the system we will use. Historically these units are based on the metric system which was developed in France at the time of the French Revolution.
Base quantity | Name | Symbol |
---|---|---|
length | metre | m |
mass | kilogram | kg |
time | second | s |
electric current | ampere | A |
thermodynamic temperature | kelvin | K |
amount of substance | mole | mol |
luminous intensity | candela | cd |
All physical quantities have units which can be built from the 7 base units listed in Table 1.1 (incidentally the choice of these seven was arbitrary). They are called base units because none of them can be expressed as combinations of the other six. This is similar to breaking a language down into a set of sounds from which all words are made. Another way of viewing the base units is like the three primary colours. All other colours can be made from the primary colours but no primary colour can be made by combining the other two primaries.
Unit names are always written with lowercase initials (e.g. the metre). The symbols (or abbreviations) of units are also written with lowercase initials except if they are named after scientists (e.g. the kelvin (K) and the ampere (A)). An exception to this rule is the litre, which is abbreviated as either L or l.
To make life convenient, particular combinations of the base units are given special names. This makes working with them easier, but it is always correct to reduce everything to the base units. Table 1.2 lists some examples of combinations of SI base units assigned special names. Do not be concerned if the formulae look unfamiliar at this stage—we will deal with each in detail in the chapters ahead (as well as many others)!
It is very important that you are able to say the units correctly. For instance, the newton is another name for the kilogram metre per second squared (kg·m·s−2), while the kilogram metre squared per second squared (kg·m2·s−2) is called the joule.
Quantity | Formula | Unit Expressed
in |
Name of |
---|---|---|---|
Base Units | Combination | ||
Force | m·a | kg·m·s−2 | N (newton) |
Frequency | s−1 | Hz (hertz) | |
Work & Energy | F·s | kg·m2·s−2 | J (joule) |
Electrical Potential | W/A | kg·m2·s−3·A−1 | V (volt) |
Another important aspect of dealing with units is the prefixes that they sometimes have (prefixes are words or letters written in front that change the meaning). The kilogram (kg) is a simple example: 1 kg is 1000 g or . Grouping the 103 and the g together we can replace the 103 with the prefix k (kilo). Therefore the k takes the place of the 103. Incidentally the kilogram is unique in that it is the only SI base unit containing a prefix.
There are prefixes for many powers of 10 (Table 1.3 lists a large set of these prefixes). This is a larger set than you will need but it serves as a good reference. The case of the prefix symbol is very important. Where a letter features twice in the table, it is written in uppercase for exponents bigger than one and in lowercase for exponents less than one. Those prefixes listed in boldface should be learned.
Prefix | Symbol | Exponent | Prefix | Symbol | Exponent |
---|---|---|---|---|---|
yotta | Y | 1024 | yocto | y | 10−24 |
zetta | Z | 1021 | zepto | z | 10−21 |
exa | E | 1018 | atto | a | 10−18 |
peta | P | 1015 | femto | f | 10−15 |
tera | T | 1012 | pico | p | 10−12 |
giga | G | 109 | nano | n | 10−9 |
mega | M | 106 | micro | µ | 10−6 |
kilo | k | 103 | milli | m | 10−3 |
hecto | h | 102 | centi | c | 10−2 |
deca | da | 101 | deci | d | 10−1 |
As another example of the use of prefixes,
can be written as 1 mg (1 milligram).
The Other Systems of Units
The remaining sets of units, although not used by us, are also internationally recognised and still in use by others. We will mention them briefly for interest only.
CGS and MKS Units
In this system the basic measure of length is the centimetre, weight is in grams and time is in seconds. Later the metre is replaced the centimetre and the kilogram replaced the gram. The Second has remained the basic unit of time throughout. This is a simple change but it means that all units derived from these two are changed. For example, the units of force and work are different. These units are used most often in astrophysics and atomic physics.
When electromagnetism comes into play, there are three CGS systems, adapted to the fundamental equations each theory views as basic: The electric CGS, the magnetic CGS, and the combined Gaussian. The latter has the advantage that corresponding electric and magnetic phenomena have the same units and related equations.
It has the additional advantage that there is only one natural constant in the equations, the speed of light, where the SI system has two. And experience, i.e. measurements, has shown that there is only one constant. So the Gaussion system is a bit more 'right'.
These unit systems also show that the choice of base units is arbitrary. In SI, there is a base unit for the current, the ampere [A], derived from it the unit of charge, coulomb [C]. The Gaussian system does without a dedicated unit for electricity. It simply defines the factor in the law of force between two charged particles as one - and lo, the unit C disappears; the esu (electrostatic unit) can be derived from g, cm, s - the C cannot, it is As, and A is basic.
[The same could be done with mass, leading to kg vanishing, just by setting the gravitational constant in Newton's law to one. kg would then be replaced by a combination of m and s.
Imperial Units
These units (as their name suggests) stem from the days when the Roman Empire decided measures. Some of these were later altered by local rulers. As a result, different countries used different base units for each quantity (except for time). The British abandoned the Roman measurement and money system in 1972. There were 12 pennies or denaries in a shilling or solidus, and 20 shillings in a pound or libra ergo there were 240 'old pennies' and are now 100 new pennies in the pound sterling or GBP - which large unit was unchanged. The British also used both avoirdupois and troy weight and other capricious local measures, but following its integration in the EU, Britain now officially use decimal SI units for all measurements.
Although the British once used an imperial metric system similar to that in use in the US, it is important to know that there are some differences, because the colonists made certain incorrect assumptions, such as that because there were 16 ounces in a pound weight, there were also 16 fluid ounces in a pint of liquid, when the Romans and British defined 20 fl oz. This matters, because during World War II, for example, great fraud was perpetrated by the British selling the smaller American gallons (8 pints) at the price for the larger British measure!
The decimal metric system was invented in France in 1791, following the French revolution. This later became the MKS (Meter/Kilogram/Second) system and is now the System International (SI) system, which is still close that early French system. Using different units in different places would make effective scientific communication very difficult. That is why the scientific community has adopted SI units as its internationally agreed upon standard. Therefore the SI is overwhelmingly predominant for nearly all international scientific and technical use.
Natural Units
This is the most sophisticated choice of units. Here the most fundamental discovered quantities (such as the speed of light) are set equal to 1. The argument for this choice is that all other quantities should be built from these fundamental units. This system of units is used in high energy physics and quantum mechanics.
The Importance of Units
Without units much of our work as scientists would be meaningless. We need to express our thoughts clearly and units give meaning to the numbers we calculate. Depending on which units we use, the numbers are different (e.g. 3.8 m and 3800 mm actually represent the same length). Units are an essential part of the language we use. Units must be specified when expressing physical quantities. In the case of the curtain example at the beginning of the chapter, the result of a misunderstanding would simply have been an incorrect amount of material cut. However, sometimes such misunderstandings have catastrophic results. Here is an extract from a story on CNN's website:
NASA: Human error caused loss of Mars orbiter November 10, 1999
WASHINGTON (AP) -- Failure to convert English measures to metric values caused the loss of the Mars Climate Orbiter, a spacecraft that smashed into the planet instead of reaching a safe orbit, a NASA investigation concluded Wednesday. The Mars Climate Orbiter, a key craft in the space agency's exploration of the red planet, vanished after a rocket firing September 23 that was supposed to put the spacecraft on orbit around Mars. An investigation board concluded that NASA engineers failed to convert English measures of rocket thrusts to newton, a metric system measuring rocket force. One English pound of force equals 4.45 newtons. A small difference between the two values caused the spacecraft to approach Mars at too low an altitude and the craft is thought to have smashed into the planet's atmosphere and was destroyed. The spacecraft was to be a key part of the exploration of the planet. From its station about the red planet, the Mars Climate Orbiter was to relay signals from the Mars Polar Lander, which is scheduled to touch down on Mars next month. ``The root cause of the loss of the spacecraft was a failed translation of English units into metric units and a segment of ground-based, navigation-related mission software," said Arthus Stephenson, chairman of the investigation board. Reported by CNN at http://www.cnn.com/TECH/space/9911/10/orbiter.02/
This story illustrates the importance of being aware that different systems of units exist. Furthermore, we must be able to convert between systems of units!
Choice of Units
There are no wrong units to use, but a clever choice of units can make a problem look simpler. The vast range of problems makes it impossible to use a single set of units for everything without making some problems look much more complicated than they should. We can't easily compare the mass of the sun and the mass of an electron, for instance. This is why astrophysicists and atomic physicists use different systems of units.
We won't ask you to choose between different unit systems. For your present purposes the SI system is perfectly sufficient. In some cases you may come across quantities expressed in units other than the standard SI units. You will then need to convert these quantities into the correct SI units. This is explained in the next section.
How to Change Units-- the "Multiply by 1" Technique
Also known as fractional dimensional analysis, the technique involves multiplying a labeled quantity by a conversion ratio, or knowledge of conversion factors. First, a relationship between the two units that you wish to convert between must be found. Here's a simple example: converting millimetres (mm) to metres (m)-- the SI unit of length. We know that there are 1000 mm in 1 m which we can write as
Now multiplying both sides by
we get
which simply gives us
This is the conversion ratio from millimetres to metres. You can derive any conversion ratio in this way from a known relationship between two units. Let's use the conversion ratio we have just derived in an example:
Question: Express 3800 mm in metres.
Answer:
Note that we wrote every unit in each step of the calculation. By writing them in and cancelling them properly, we can check that we have the right units when we are finished. We started with mm and multiplied by
This cancelled the mm leaving us with just m, which is the SI unit we wanted! If we wished to do the reverse and convert metres to millimetres, then we would need a conversion ratio with millimetres on the top and metres on the bottom.
It is helpful to understand that units cancel when one is in the numerator and the other is in the denominator. If the unit you are trying to cancel is on the top, then the conversion factor that you multiply it with must be on the bottom.
This same technique can be used to not just to convert units, but can also be used as a way to solve for an unknown quantity. For example: If I was driving at 65 miles per hour, then I could find how far I would go in 5 hours by using as a conversion factor.
This would look like
This would yield a result of 325 miles because the hours would cancel leaving miles as the only unit.
Practice Problem
Problem: Convert 3 millennia into seconds.
Most people don't know how many seconds are in a millennium, but they do know enough to solve this problem. Since we know 1000 years = 1 millennium, 1 year = about 365.2425 days, 1 day = 24 hours, and 1 hour = 3600 seconds we can solve this problem by multiplying by one many times.
How Units Can Help You
We conclude each section of this book with a discussion of the units most relevant to that particular section. It is important to try to understand what the units mean. That is why thinking about the examples and explanations of the units is essential.
If we are careful with our units then the numbers we get in our calculations can be checked in a 'sanity test'.
What is a 'sanity test'?
This isn't a special or secret test. All we do is stop, take a deep breath, and look at our answer. Sure we always look at our answers—or do we? This time we mean stop and really look—does our answer make sense?
Imagine you were calculating the number of people in a classroom. If the answer you got was 1 000 000 people you would know it was wrong—that's just an insane number of people to have in a classroom. That's all a sanity check is—is your answer insane or not? But what units were we using? We were using people as our unit. This helped us to make sense of the answer. If we had used some other unit (or no unit) the number would have lacked meaning and a sanity test would have been much harder (or even impossible).
It is useful to have an idea of some numbers before we start. For example, let's consider masses. An average person has mass 70 kg, while the heaviest person in medical history had a mass of 635 kg. If you ever have to calculate a person's mass and you get 7000 kg, this should fail your sanity check—your answer is insane and you must have made a mistake somewhere. In the same way an answer of 0.00001 kg should fail your sanity test.
The only problem with a sanity check is that you must know what typical values for things are. In the example of people in a classroom you need to know that there are usually 20-50 people in a classroom. Only then do you know that your answer of 1 000 000 must be wrong. Here is a table of typical values of various things (big and small, fast and slow, light and heavy—you get the idea):
|
(NOTE TO SELF: Add to this table as we go along with examples from each section)
Note that you do not have to memorize this table. However, read it so that you can refer to it when you do a calculation.
Temperature
In everyday life, many people measure temperatures in Celsius. But in physics, we prefer to use the Kelvin scale which starts at absolute zero(−273.15 °C).
As we all know, Celsius temperatures can be negative. This might suggest that any number is a valid temperature. In fact, the temperature of a gas is a measure of the average kinetic energy of the particles that make up the gas. As we lower the temperature so the motion of the particles is reduced until a point is reached where all motion ceases. The temperature at which this occurs is called absolute zero. There is no physically possible temperature colder than this. In Celsius, absolute zero is at -273.15 °C. In Kelvin, the ordinary freezing point of water 0 °C is therefore 273.15 K
Physicists have defined a new temperature scale called the Kelvin scale. According to this scale absolute zero is at 0 K and negative temperatures are not allowed. The size of one unit kelvin is exactly the same as that of one unit degree Celsius. This means that a change in temperature of 1 kelvin is equal to a change in temperature of 1 degree Celsius—the scales just start in different places. Think of two ladders with steps that are the same size but the bottom most step on the Celsius ladder is labelled -273.15, while the first step on the Kelvin ladder is labelled 0. There are still 100 'steps' or degrees (Celsius or Kelvin) between the points where water freezes and boils when it is at at 1.0 atmosphere of pressure (water boils at lower temperatures if the air pressure is lowerO.
|- |- water boils ---> |----| 100 degrees Celsius |----| 373 kelvin |- |- . . . |- |- ice melts ---> |----| 0 degrees Celsius |----| 273 kelvin |- |- . . . |- |- |- |- absolute zero ---> |----| -273 degrees Celsius |----| 0 kelvin
(NOTE TO SELF: Come up with a decent picture of two ladders with the labels—water boiling and freezing—in the same place but with different labelling on the steps!)
This makes the conversion from kelvin to degree Celsius and back very easy. To convert from degrees Celsius to kelvins add 273.15. To convert from kelvins to degrees Celsius subtract 273.15. Representing the Kelvin temperature by TK and the Celsius temperature by T°C,
Converting between kelvin and Celsius is additive—so a difference in temperature of 1 degree Celsius is equal to a difference of 1 kelvin. The majority of conversions between units are multiplicative. For example, to convert from metres to millimetres we multiply by 1000. Therefore a change of 1 m is equal to a change of 1000 mm.
Although it seems as though there is not much reason for the scientific community to use the Kelvin scale over the Celsius scale, there is actually a very remarkable difference in using the Kelvin scale other than the reminding effect stated above. It happens that the idealised form of some materials varies proportionately according to the Kelvin scale, such that some required values can be simply found by multiplication and division. In such calculations, it is just a chore to use the Celsius scale.
Fahrenheit Scale
Fahrenheit is a temperature scale previously used in meteorology until about 1970, and which is still widely used in the USA. It was devised by a German physicist in 1724. He needed a scale that went below the freezing point of water, and used a mixture of ice, water, and ammonium chloride which freezes at a much lower temperature.
In this scale, the freezing point of water (0 °C) is 32 degrees Fahrenheit (32 °F) and the boiling point (100 °C) is 212 °F, placing the boiling and freezing points of water exactly 180 degrees apart. A temperature interval of one degree Fahrenheit is an interval of 5⁄9 of a degree Celsius. The Fahrenheit and Celsius scales coincide at −40 degrees (i.e. −40 °F and −40 °C describe the same temperature).
To convert Celsius to Fahrenheit:
1) Take your number in Celsius and multiply by 9.
2) Divide the result of step 1 by 5.
3) Add 32 to the result of step 2.
Converting Fahrenheit to Celsius:
1) Take your number in Fahrenheit and subtract 32
2) Multiply the result of step 1 by 5.
3) Divide the result of step 2 by 9.
Rankine Scale
Absolute zero is −459.67 °F. The Rankine temperature scale was created to use degrees the same size as those of the Fahrenheit scale, such that a temperature difference of one degree Rankine (1 °R) is the same as a temperature difference of 1 °F, but with absolute zero being 0 °R.
Scientific Notation, Significant Figures and Rounding
If you are only sure of say, both digits of a two-digit number, and put it in a formula and get a long series of numbers to the right of the decimal place, then those digits are probably not very accurate. This is the idea of significant figures.
Take 10 and divide by 3. If you are not sure that the number 10 is perfectly accurate, then you do not need to write down 3.333... and can get away with something like 3.3 or 3.33
(NOTE TO SELF: still to be written)
The accuracy of a measurement using significant figures is represented by the number of digits that it contains. A number is said to have the number of significant figures equal to the number of digits in the number not including leading 0s or trailing 0s unless there is a decimal point. The table below contains a list of numbers and how many significant digits each contains.
As you may have noted, some numbers cannot be shown in proper significant figure notation without the use of scientific notation. For example, the number 1000 can only be shown to have 1 or 4 or more significant digits by the inclusion of a decimal point. However, by rewriting 1000 as any number of significant digits may be added by simply add additional 0s after the decimal point. Sometimes you may be asked to determine the number of significant figures in a given number. There are three rules to determine what numerals are significant.
E notationVery large numbers such as the speed of light (the C part of Einstein's famous ) are difficult to write accurately. We could write 300,000,000 m/sec, , 300 million meters per second or some such. There is a much better way! We simply separate the number (coefficient) part 3 from its multiplier 00000000 base! But be careful, there is an Elephant trap here, and it is that in scientific notation the number is always expressed as a decimal fraction with a maximum value of 1.0, (in this case =0.3) so the multiplier part is one bigger than you might expect! is the same as 0.3E9. (Because there are a total of nine digits after the decimal point) A tiny dust particle might weigh as little as 0.000 000 000 678 kg.! This time we shift the decimal point 9 places to the right so the number (678) has a negative base so our weight is written as 0.678E-9 kg. Now is that not a whole lot easier to write and understand? That is why many scientific calculators and most spread-sheets allow input and display in E notation format. Commas and pointsMost English speaking people use the comma [ , ] to separate thousands and the dot (ful-stop, or point) [ . ] for the decimal indicator. Europeans often use these the other way around. Many spread-sheets allow both, but it is just one more complexity you need to know about! ConclusionIn this chapter we have discussed the importance of units. We have discovered that there are many different units to describe the same thing, although you should stick to SI units in your calculations. We have also discussed how to convert between different units. This is a skill you must acquire. Waves and Wavelike MotionWaves and Wavelike MotionWaves occur frequently in nature. The most obvious examples are waves in water on a dam, in the ocean, or in a bucket, but sound waves and electromagnetic waves are other, less visible examples. We are most interested in the properties that waves have. All waves have the same basic properties, so by studying waves in water we can transfer our knowledge and predict how other types of waves will behave. Waves are associated with energy. As the waves move, they carry energy from one point to another in space. It is true for water waves as well. You can see the wave energy working while a ship drifts along the wave in rough sea. The most spectacular example is the enormous amount of energy we receive from the sun in the form of light and heat, which are transmitted as electromagnetic waves - not even requiring a medium to propagate. wave was being discovered by Sir Reagan Lulu Wokoz Simple Harmonic MotionSimple Harmonic motion is a wavelike motion. It is considered wavelike because the graph of time vs. displacement from the equilibrium position is a sine curve. An example of simple harmonic motion is a mass oscillating on a spring. It will be hard to understand the forces involved this early in the course that cause the motion to simple harmonic, but it is still possible to look at a mass oscillating on a spring and understand that it is indeed simple harmonic. When a mass is oscillating on a spring, the further the string stretches, the slower the mass will be moving. Then the mass reaches a point where the string won't stretch any further, so it quits moving and then it reverses direction. As it moves closer to the equilibrium position is moves faster. What are waves?Waves are phenomena that everyone experiences constantly; water waves, sound waves, light waves, human waves when the home team scores... the list goes on. When asked what makes a wave a wave, the most common responses would probably be that a wave is something that moves, or propagates, or perhaps that it is something that repeats over and over again. These properties do capture the essential qualities of waves. Now we must determine these properties quantitatively, and discover what governs their behavior. Generally, a wave is defined as any phenomenon which can be modeled by a function of the form where the -vector represents a position in space, and represents a time, and the -vector and omega are both constants. Don't be intimidated by the vectors in the argument - most of our time at first will be spent on one-dimensional waves. If the wave is in only one spatial dimension 'x', for instance a wave travelling on a taut string, it can be written simply as . Any function of this form "propagates" along the direction over time. As time increases, the argument of the function increases; over time the form of the function effectively advances through space. Try coming up with functions of this form, and plot them at time , then plot them again at a later time. This progressive property will become obvious. Try to figure out the velocity with which your function advances! (we will study this later) The negative sign in front of the time term causes the wave to propagate in the direction defined as positive (if that seems confusing, try plotting more functions over time, and examine the results). If you replace the negative with a positive (or instead consider a negative value of omega), the wave will propagate in the negative direction. A very special and important case of a wave is the mathematical function , or in one dimension, . This is a sinusoidal wave – it oscillates up and down infinitely in both directions, and moves as time progresses. I mentioned that waves have the quality of repeating over and over, the quality of periodicity. However, many functions of the form mentioned above do not seem to repeat. However, you will find that ALL waves can be decomposed into a sum of many of these simple, infinitely repeating waves when you learn about Fourier transformations. More than any other concept, physicists are finding that waves characterize the structure of the universe at every scale imaginable. As you learn about the physics of waves in everyday life, keep an open mind towards finding waves and wave behavior everywhere you turn. Let's consider a very well-known case of a wave phenomenon: water waves. Waves in water consist of moving peaks and troughs. A peak is a place where the water rises higher than when the water is still and a trough is a place where the water sinks lower than when the water is still. So waves have peaks and troughs. This could be our first property for waves. The following diagram shows the peaks and troughs on a wave. In physics we try to be as quantitative as possible. If we look very carefully we notice that the height of the peaks above the level of the still water is the same as the depth of the troughs below the level of the still water. Waves are repetitions of physical quantity in a periodic manner, carrying energy in the process. The water waves, for example, can be visualized to repeat any of the physical quantities like "peaks", "troughs", "potential energy" or "kinetic energy". Even, we can visualize water waves as the motion of disturbance (energy). It is the energy aspect of waves that is central to the understanding of different types of waves, many of which are not visible. Looking closely at the water wave, we can recognize that crests and troughs basically represent of extreme potential and kinetic energies in addition to representing rise and fall of water from the still level. At the peak, energy is only potential, whereas energy is only kinetic at the trough. Similarly, propagation of electromagnetic wave is associated with repetitions of magnetic and electric field in space with certain periodicity. As existence of electrical or magnetic fields does not require any medium, electromagnetic waves can move even in the absence of any medium. Characteristics of Waves: AmplitudeWe use symbols agreed upon by convention to label the characteristic quantities of the waves. The characteristic height of a peak and depth of a trough is called the amplitude of the wave. The vertical distance between the bottom of the trough and the top of the peak is twice the amplitude. To put it simply, the amplitude is the distance of the wave from the medium, to the crest or trough Worked Example 1 Question: (NOTE TO SELF: Make this a more exciting question) The height of the wave from the medium is 2m. What is the distance from the peak to the trough. What
The amplitude is 2m. (Read above paragraph to know why). The distance from the peak to trough is 4m. Characteristics of Waves : WavelengthLook a little closer at the peaks and the troughs. The distance between two adjacent (next to each other) peaks is the same no matter which two adjacent peaks you choose. So there is a fixed distance between the peaks. Similarly, you'll notice that the distance between two adjacent troughs is the same no matter which two troughs you look at. But, more importantly, its is the same as the distance between the peaks. This distance which is a characteristic of the wave is called the wavelength. Waves have a characteristic wavelength. The symbol for the wavelength is the Greek letter lambda, . The wavelength is the distance between any two adjacent points which are in phase. Two points in phase are separate by an integer (0,1,2,3,...) number of complete wave cycles. They don't have to be peaks or trough but they must be separated by a complete number of waves. Characteristics of Waves : PeriodNow imagine you are sitting next to a pond and you watch the waves going past you. First one peak, then a trough and then another peak. If you measure the time between two adjacent peaks you'll find that it is the same. Now if you measure the time between two adjacent troughs you'll find that its always the same, no matter which two adjacent troughs you pick. The time you have been measuring is the time for one wavelength to pass by. We call this time the period and it is a characteristic of the wave. The period of the wave is denoted with the symbol . Characteristics of Waves : FrequencyThere is another way of characterising the time interval of a wave. We timed how long it takes for one wavelength to go past. We could also turn this around and say how many waves go by in 1 second. We can easily determine this number, which we call the frequency and denote f. To determine the frequency, how many waves go by in 1s, we work out what fraction of a waves goes by in 1 second by dividing 1 second by the time it takes T. If a wave takes 1/2 a second to go by then in 1 second two waves must go by. . The unit of frequency is the Hz or s−1. Waves have a characteristic frequency.
|